Functional modulation of a G Protein-Coupled Receptor conformational landscape in a lipid bilayer
Thesis and Post-Doc of Marina Casiraghi (2013-2017)
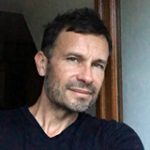
Laurent J.
Catoire
Research Director
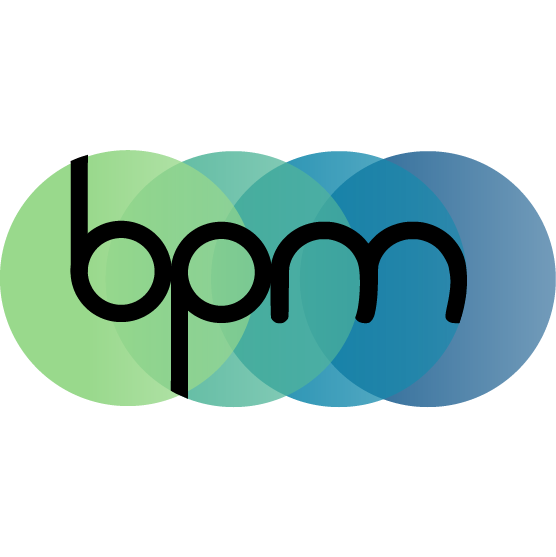
Miri
Garrigues
pHD STUDENT
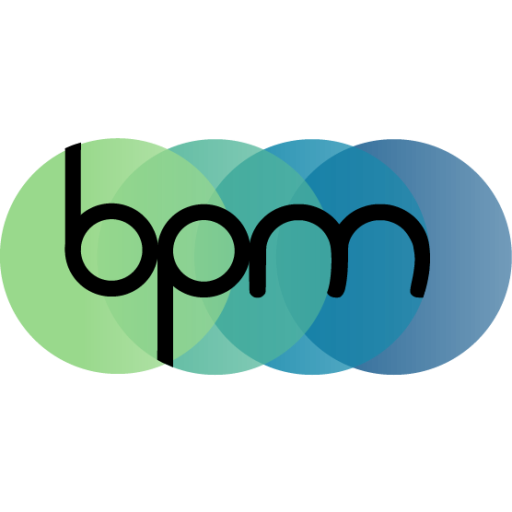
Claudia
Zilian
Post-doc
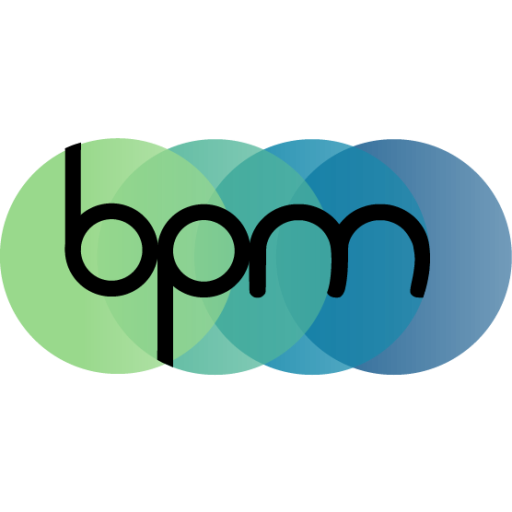
Nahya
Nguyen Armengaud
M2 Student
Figure1: Incorporation of fully active perdeuterated BLT2 in a lipid nanodiscs from E. coli inclusion bodies.
Schematic diagram of the method to obtain high amounts of recombinant fully active and stable u-2H, 13CH3-designed-probes GPCRs associated to lipid nanodiscs in solution.
i) Production of the GPCR to inclusion bodies (IBs) in E. coli in a 100%-D2O solution.
ii) IBs are subsequently solubilized and the receptor purified in a SDS-detergent solution, leading to a secondary-structured folded GPCR.
iii) A complete folding is achieved by incubating the receptor in the presence of APols and lipids.
iv) APol-trapped GPCR transferred to NLBs: substitution of APol molecules at the transmembrane surface of the receptor is achieved using mild detergent solutions in the presence of cholesteryl hemisuccinate (CHS) and divalent cations. The reconstitution in NLBs is realized with the receptor immobilized on the Ni-charged resin. The NLBs were composed of partially deuterated di-myristoyl-phosphatidylcholine (DMPC) and protonated cholesteryl hemisuccinate (CHS) as the lipids and stabilized by the protonated lipoprotein MSP1D1. The reconstitution conditions used, i.e. a large excess in MSP protein, have been shown to systematically provide monomeric receptor preparations for all GPCRs considered so far [14-19]. Accordingly, the samples so obtained were homogeneous based on size exclusion chromatography and negative-stained images obtained by electron microscopy (see sup info in ref 20).
Figure 2: Incorporation of fully active perdeuterated BLT2 in a lipid nanodiscs from E. coli inclusion bodies.
(a) Fluorescence anisotropy-monitored stoichiometric titration of a 15 μM solution of LTB4-568 by BLT2-containing nanodiscs (closed circles) or empty discs (open circles). A molar binding ratio of 0.95 is inferred from this plot, indicating that 95% of the BLT2 receptor is functional.
(b) Fluorescence anisotropy-monitored dose-response binding of LTB4-568 to empty discs (open circles) or BLT2-containing nanodiscs (closed circles).
(c) Fluorescence anisotropy-monitored competition experiments. LTB4-568 was displaced from its binding site in the presence of increasing concentrations in LTB4 (closed circles), in the presence of BLT2-specific ligand LY2552833 (open squares) or the BLT1-specific antagonist U75302 (closed squares).
(d) Variations of the fluorescence anisotropy signal of LTB4-568 as a function of time at two different temperatures in the presence of empty discs (open and closed squares) or BLT2-containing nanodiscs (open and closed circles).
(e) GTPγS binding to Gαiβγ catalyzed by empty or BLT2-containing nanodiscs in the absence of ligand or in the presence of 1µM LTB4 or LY2552833.
(f) LTB4 or LY2552833 saturation of GTPγS binding to Gαiβγ in the presence of empty discs (open and closed squares) or BLT2 containing nanodiscs (open and closed circles). Inset: time-dependent activation of the G protein catalyzed by BLT2 containing discs or empty discs in the absence or presence of LTB4. In all cases (a-f), data are represented as mean ±SD of three representative experiments.
Thanks to a perdeuteration and a fine tuned biochemistry, we were able to obtained NMR data at a resolution never observed in the field. This provides direct evidence that BLT2 explores a complex landscape that includes four different conformational states for the unliganded receptor (Fig. 3). The relative distribution of the different states is modulated by ligands and the sterol content of the membrane, in parallel with the changes in the ability of the receptor to activate its cognate G protein. This demonstrates a conformational coupling between the agonist and the membrane environment that is likely to be fundamental for GPCR signaling.
Figure 3: BLT2 conformational landscape.
(a) Impact of the sterol CHS content and ligands on BLT2 conformational landscape and constitutive activity. Zooms in the [δ1–13CH3]-Ile229 region of superimposed 2D NMR 1H,13C SOFAST-methyl-HMQC/TROSY spectra of u-2H,12C Ile-[δ1–13CH3], 12C Met-[ε-13CH3] wild-type BLT2 in nanodiscs in the unliganded (in black) and liganded states (in red) at low (top and bottom left) or high (top and bottom right) CHS/DMPC ratios. Agonist and partial agonist refer to LTB4 and LY2552833, respectively. On both edges are indicated the evolution of the active-like state IV upon an increase of the sterol content. These experiments indicate that the sterol has a positive allosteric effect on BLT2 activation as confirmed by GTP-to-G protein binding experiments carried out in the same conditions (see ref 20).
(b) Schematic summary to indicate that a similar evolution of the conformational ensemble by varying the sterol content in the absence or presence of ligands has been observed for transmembrane residues Met 105 (Helix III) and Ile 229 (Helix VI) which suggests a concerted mechanism between two distinct regions in the transmembrane part of the receptor.
(c) The communicating vessel observed between the different sub-states in BLT2 conformational ensemble allows to propose a simple and preliminary model of activation for this receptor. Based on this model, the addition of the sterol decreases the overall free energy difference between states II and IV by about 2 kcal/mol. This represents a variation of the equilibrium constant in favor of the active state by a factor ~30. These 2 kcal/mol are enough to modify interactions between TM α-helices, estimated to be of the order of 1-10 kcal/mol for a pair of helices [21], but not to destabilize the α-secondary structure of a TM helix, which would require 20-30 kcal/mol [22]. In other words, the addition of the sterol may facilitate a reorganization of the helix bundle in favor of the active or active-like state (in the absence of a G protein).
In collaboration with
Jean-Louis Banères
(IBMM, Montpellier)
(Marjorie Damian, Jacky Marie)
Eric Guittet
(ICSN, Gif/Yvette)
(Ewen Lescop, Nelly Morellet)
Bibliography (in blue from the lab):
- Bokoch et al. (2010) Nature, 463, 108. (Pubmed)
- Liu et al. (2012) Science, 335, 1106. (Pubmed)
- Kofuku et al. (2012) Nat Commun, 3, 1045. (Pubmed)
- Nygaard et al. (2013) Cell, 152, 532. (Pubmed)
- Kim et al. (2013) J Am Chem Soc, 135, 9465. (Pubmed)
- Manglik et al. (2015) Cell, 161, 1101. (Pubmed)
- Sounier et al. (2015) Nature, 2015, 524, 375. (Pubmed)
- Kofuku et al. (2015) Angew Chem Int Ed, 53, 13376. (Pubmed)
- Gautier et al. (2010) Nat Struct Mol Biol, 17, 768. (Pubmed)
- Isogai et al. (2016)Nature, 530, 237. (Pubmed)
- Chung et al. (2012) J Biol Chem, 287, 36305. (Pubmed)
- Popot (2010) Annu Rev Biochem, 79, 737. (Pubmed)
- Ritchie et al. (2009) Methods Enzymol, 464, 211. (Pubmed)
- Popot et al. (2011) Annu Rev Biophys, 40, 379. (Pubmed)
- Whorton et al. (2007) Proc Natl Acad Sci USA, 104, 7682. (Pubmed)
- Kuszak et al. (2009) J Biol Chem, 284, 26732. (Pubmed)
- Inagaki et al. (2012) J Mol Biol, 417, 95. (Pubmed)
- Damian et al. (2012) J Biol Chem, 287, 3630. (Pubmed)
- El Moustaine et al. (2012) Proc Natl Acad Sci USA, 109, 16342. (Pubmed)
- Casiraghi et al. (2016) J Am Chem Soc, 138, 11170. (Pubmed)
- Popot & Engelman (1990) Biochemistry, 29, 4031. (Pubmed)
- Lemmon & Engelman (1994) Q Rev Biophys, 27, 157. (Pubmed)